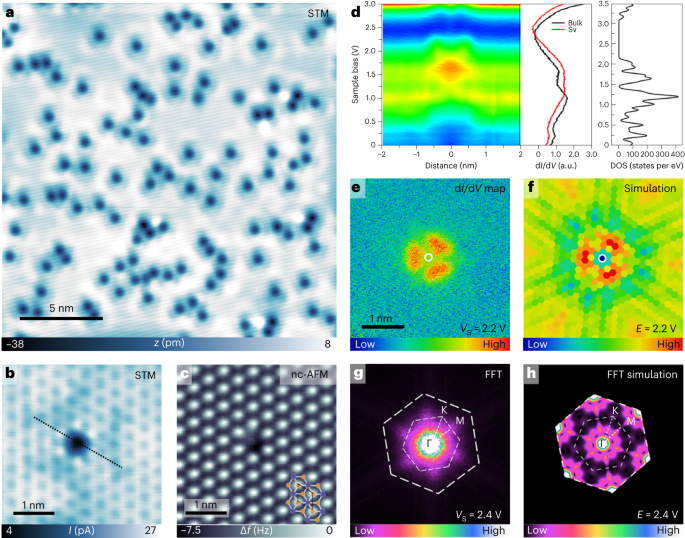
Fuechsle, M. et al. A single-atom transistor. Nat. Nanotechnol. 7, 242–246 (2012).
Huff, T. et al. Binary atomic silicon logic. Nat. Electron. 1, 636–643 (2018).
Achal, R. et al. Lithography for robust and editable atomic-scale silicon devices and memories. Nat. Commun. 9, 2778 (2018).
Kalff, F. E. et al. A kilobyte rewritable atomic memory. Nat. Nanotechnol. 11, 926–929 (2016).
Amlani, I. et al. Digital logic gate using quantum-dot cellular automata. Science 284, 289–291 (1999).
Imre, A. et al. Majority logic gate for magnetic quantum-dot cellular automata. Science 311, 205–208 (2006).
Kim, D. et al. Quantum control and process tomography of a semiconductor quantum dot hybrid qubit. Nature 511, 70–74 (2014).
Fölsch, S., Martínez-Blanco, J., Yang, J., Kanisawa, K. & Erwin, S. C. Quantum dots with single-atom precision. Nat. Nanotechnol. 9, 505–508 (2014).
Du, A. et al. Dots versus antidots: computational exploration of structure, magnetism, and half-metallicity in boron-nitride nanostructures. J. Am. Chem. Soc. 131, 17354–17359 (2009).
Mitterreiter, E. et al. The role of chalcogen vacancies for atomic defect emission in MoS2. Nat. Commun. 12, 3822 (2021).
Flindt, C., Mortensen, N. A. & Jauho, A.-P. Quantum computing via defect states in two-dimensional antidot lattices. Nano Lett. 5, 2515–2518 (2005).
Pedersen, T. G. et al. Graphene antidot lattices: designed defects and spin qubits. Phys. Rev. Lett. 100, 136804 (2008).
Besteiro, L. V., Kong, X.-T., Wang, Z., Hartland, G. & Govorov, A. O. Understanding hot-electron generation and plasmon relaxation in metal nanocrystals: quantum and classical mechanisms. ACS Photon. 4, 2759–2781 (2017).
Zhang, H. et al. Large-scale mesoscopic transport in nanostructured graphene. Phys. Rev. Lett. 110, 066805 (2013).
Clavero, C. Plasmon-induced hot-electron generation at nanoparticle/metal-oxide interfaces for photovoltaic and photocatalytic devices. Nat. Photon. 8, 95–103 (2014).
Goldman, V. J. & Su, B. Resonant tunneling in the quantum hall regime: measurement of fractional charge. Science 267, 1010–1012 (1995).
Maasilta, I. J. & Goldman, V. J. Tunneling through a coherent ‘quantum antidot molecule’. Phys. Rev. Lett. 84, 1776–1779 (2000).
Sim, H.-S. et al. Coulomb blockade and kondo effect in a quantum Hall antidot. Phys. Rev. Lett. 91, 266801 (2003).
Park, M., Harrison, C., Chaikin, P. M., Register, R. A. & Adamson, D. H. Block copolymer lithography: periodic arrays of ~1011 holes in 1 square centimeter. Science 276, 1401–1404 (1997).
Sinitskii, A. & Tour, J. M. Patterning graphene through the self-assembled templates: toward periodic two-dimensional graphene nanostructures with semiconductor properties. J. Am. Chem. Soc. 132, 14730–14732 (2010).
Sandner, A. et al. Ballistic transport in graphene antidot lattices. Nano Lett. 15, 8402–8406 (2015).
Jessen, B. S. et al. Lithographic band structure engineering of graphene. Nat. Nanotechnol. 14, 340–346 (2019).
Khajetoorians, A. A., Wegner, D., Otte, A. F. & Swart, I. Creating designer quantum states of matter atom-by-atom. Nat. Rev. Phys. 1, 703–715 (2019).
Gomes, K. K., Mar, W., Ko, W., Guinea, F. & Manoharan, H. C. Designer Dirac fermions and topological phases in molecular graphene. Nature 483, 306–310 (2012).
Slot, M. R. et al. Experimental realization and characterization of an electronic Lieb lattice. Nat. Phys. 13, 672–676 (2017).
Kempkes, S. N. et al. Design and characterization of electrons in a fractal geometry. Nat. Phys. 15, 127–131 (2019).
Drost, R., Ojanen, T., Harju, A. & Liljeroth, P. Topological states in engineered atomic lattices. Nat. Phys. 13, 668–671 (2017).
Xu, J. et al. Quantum antidot formation and correlation to optical shift of gold nanoparticles embedded in MgO. Phys. Rev. Lett. 88, 175502 (2002).
Liu, Y., Xu, F., Zhang, Z., Penev, E. S. & Yakobson, B. I. Two-dimensional mono-elemental semiconductor with electronically inactive defects: the case of phosphorus. Nano Lett. 14, 6782–6786 (2014).
Nguyen, G. D. et al. 3D imaging and manipulation of subsurface selenium vacancies in PdSe2. Phys. Rev. Lett. 121, 086101 (2018).
Liu, M., Nam, H., Kim, J., Fiete, G. A. & Shih, C.-K. Influence of nanosize hole defects and their geometric arrangements on the superfluid density in atomically thin single crystals of indium superconductor. Phys. Rev. Lett. 127, 127003 (2021).
Li, X. et al. Ordered clustering of single atomic Te vacancies in atomically thin PtTe2 promotes hydrogen evolution catalysis. Nat. Commun. 12, 2351 (2021).
Zhussupbekov, K. et al. Imaging and identification of point defects in PtTe2. npj 2D Mater. Appl. 5, 14 (2021).
Leo, G., Fabian, M., Nikolaj, M., Peter, L. & Gerhard, M. The chemical structure of a molecule resolved by atomic force microscopy. Science 325, 1110–1114 (2009).
Barja, S. et al. Identifying substitutional oxygen as a prolific point defect in monolayer transition metal dichalcogenides. Nat. Commun. 10, 3382 (2019).
Schuler, B. et al. How substitutional point defects in two-dimensional WS2 induce charge localization, spin–orbit splitting, and strain. ACS Nano 13, 10520–10534 (2019).
Cochrane, K. A. et al. Spin-dependent vibronic response of a carbon radical ion in two-dimensional WS2. Nat. Commun. 12, 7287 (2021).
Guo, G. Y. & Liang, W. Y. The electronic structures of platinum dichalcogenides: PtS2, PtSe2 and PtTe2. J. Phys. C: Solid State Phys. 19, 995 (1986).
Aghajanian, M. et al. Resonant and bound states of charged defects in two-dimensional semiconductors. Phys. Rev. B 101, 081201 (2020).
Fang, H. et al. Electronic self-passivation of single vacancy in black phosphorus via ionization. Phys. Rev. Lett. 128, 176801 (2022).
Schuler, B. et al. Large spin-orbit splitting of deep in-gap defect states of engineered sulfur vacancies in monolayer WS2. Phys. Rev. Lett. 123, 076801 (2019).
Gross, L. et al. Investigating atomic contrast in atomic force microscopy and Kelvin probe force microscopy on ionic systems using functionalized tips. Phys. Rev. B 90, 155455 (2014).
Cai, Y., Ke, Q., Zhang, G., Yakobson, B. I. & Zhang, Y.-W. Highly itinerant atomic vacancies in phosphorene. J. Am. Chem. Soc. 138, 10199–10206 (2016).
Trevethan, T., Latham, C. D., Heggie, M. I., Briddon, P. R. & Rayson, M. J. Vacancy diffusion and coalescence in graphene directed by defect strain fields. Nanoscale 6, 2978–2986 (2014).
Ishizuka, H. & Nagaosa, N. Spin chirality induced skew scattering and anomalous Hall effect in chiral magnets. Sci. Adv. 4, eaap9962 (2018).
Fujishiro, Y. et al. Giant anomalous Hall effect from spin-chirality scattering in a chiral magnet. Nat. Commun. 12, 317 (2021).
Arh, T. et al. The Ising triangular-lattice antiferromagnet neodymium heptatantalate as a quantum spin liquid candidate. Nat. Mater. 21, 416–422 (2022).
Bezanson, J., Edelman, A., Karpinski, S. & Shah, V. B. Julia: a fresh approach to numerical computing. SIAM Rev. 59, 65–98 (2017).
Kresse, G. & Furthmüller, J. Efficient iterative schemes for ab initio total-energy calculations using a plane-wave basis set. Phys. Rev. B 54, 11169–11186 (1996).
Blöchl, P. E. Projector augmented-wave method. Phys. Rev. B 50, 17953–17979 (1994).
Perdew, J. P., Burke, K. & Ernzerhof, M. Generalized gradient approximation made simple. Phys. Rev. Lett. 77, 3865–3868 (1996).
Moellmann, J. & Grimme, S. DFT-D3 study of some molecular crystals. J. Phys. Chem. C 118, 7615–7621 (2014).
Henkelman, G., Uberuaga, B. P. & Jónsson, H. A climbing image nudged elastic band method for finding saddle points and minimum energy paths. J. Chem. Phys. 113, 9901–9904 (2000).
Giannozzi, P. et al. QUANTUM ESPRESSO: a modular and open-source software project for quantum simulations of materials. J. Phys. Condens. Matter 21, 395502 (2009).
Giannozzi, P. et al. Advanced capabilities for materials modelling with Quantum ESPRESSO. J. Phys. Condens. Matter 29, 465901 (2017).
Dal Corso, A. Pseudopotentials periodic table: from H to Pu. Comput. Mater. Sci. 95, 337–350 (2014).
- SEO Powered Content & PR Distribution. Get Amplified Today.
- PlatoData.Network Vertical Generative Ai. Empower Yourself. Access Here.
- PlatoAiStream. Web3 Intelligence. Knowledge Amplified. Access Here.
- PlatoESG. Automotive / EVs, Carbon, CleanTech, Energy, Environment, Solar, Waste Management. Access Here.
- PlatoHealth. Biotech and Clinical Trials Intelligence. Access Here.
- ChartPrime. Elevate your Trading Game with ChartPrime. Access Here.
- BlockOffsets. Modernizing Environmental Offset Ownership. Access Here.
- Source: https://www.nature.com/articles/s41565-023-01495-z
- ][p
- 07
- 1
- 10
- 100
- 11
- 110
- 12
- 121
- 13
- 14
- 15%
- 16
- 17
- 19
- 1994
- 1995
- 1996
- 1999
- 20
- 2000
- 2005
- 2006
- 2008
- 2012
- 2013
- 2014
- 2015
- 2016
- 2017
- 2018
- 2019
- 2020
- 2021
- 2022
- 22
- 23
- 24
- 25
- 26
- 27
- 28
- 29
- 2D
- 30
- 31
- 32
- 33
- 36
- 39
- 3d
- 40
- 46
- 49
- 50
- 51
- 52
- 53
- 54
- 65
- 7
- 75
- 77
- 8
- 84
- 9
- 90
- 91
- a
- advanced
- AL
- am
- an
- and
- approach
- arrangements
- article
- AS
- At
- b
- BAND
- basis
- Black
- Block
- bound
- by
- candidate
- capabilities
- carbon
- case
- cellular
- charge
- charged
- chemical
- click
- Climbing
- clustering
- coalescence
- COHERENT
- computing
- contrast
- control
- Correlation
- Creating
- deep
- density
- Design
- designed
- Designer
- Devices
- Diffusion
- digital
- DOT
- e
- E&T
- effect
- efficient
- Electronic
- electronically
- electrons
- embedded
- emission
- energy
- Engineering
- Ether (ETH)
- evolution
- experimental
- exploration
- Fields
- finding
- For
- Force
- formation
- fractional
- fresh
- from
- generation
- geometry
- giant
- Gold
- goldman
- Graphene
- Hall
- highly
- Hole
- Holes
- How
- http
- HTTPS
- Hybrid
- hydrogen
- i
- Identification
- identifying
- image
- Imaging
- in
- inactive
- influence
- interfaces
- Ionic
- Kelvin
- Kim
- Kong
- large
- large-scale
- LINK
- Liquid
- Localization
- logic
- made
- Magnetism
- Magnets
- Majority
- Manipulation
- materials
- Matter
- measurement
- mechanisms
- Memories
- Memory
- metal
- method
- Microscopy
- minimum
- modelling
- modular
- molecular
- molecule
- Nam
- nanotechnology
- Nature
- of
- on
- open source
- Open-source Software
- Oxygen
- periodic
- Peter
- platinum
- plato
- Plato Data Intelligence
- PlatoData
- Point
- points
- precise
- Precision
- probe
- process
- project
- promotes
- properties
- Quantum
- quantum computing
- Quantum dot
- Quantum dots
- Qubit
- qubits
- R
- radical
- realization
- regime
- register
- relaxation
- resolved
- response
- robust
- Role
- s
- schemes
- Scholar
- SCI
- semiconductor
- Semiconductors
- set
- shift
- Silicon
- Simple
- single
- skew
- Software
- solid
- some
- Spin
- spin qubits
- square
- State
- States
- structure
- structures
- Study
- Systems
- T
- table
- templates
- The
- their
- Through
- tips
- to
- tomography
- Tour
- toward
- transition
- transport
- understanding
- using
- Versus
- via
- W
- with
- X
- zephyrnet